Research
Emergent quantum phases of light
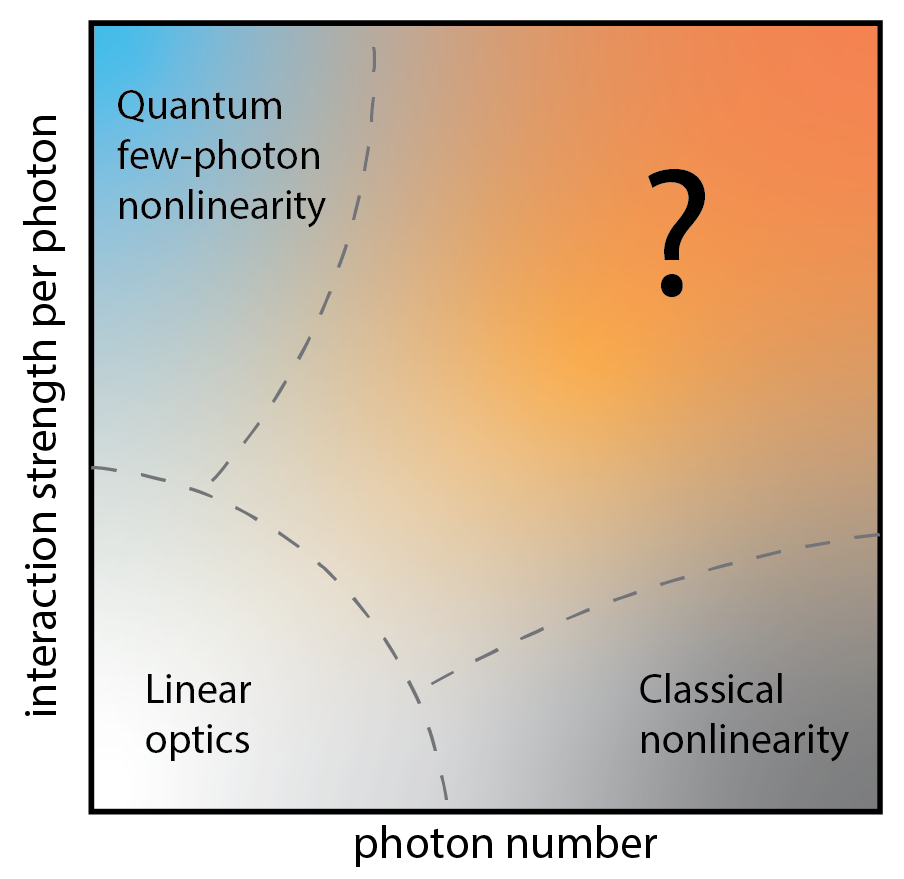
Understanding the emergent behaviour of many-body systems is one of the outstanding problems of modern science. The interplay of interaction and kinetic energies leads to strong inter-particle correlations and a rich variety of ordered phases such as superconductivity to antiferromagnetic Mott insulators. These many-body effects are typically observed in material systems such as ultracold atomic gases or electrons in solid state. However, an exciting and rapidly growing field has developed over the last two decades motivated by a fascinating question: Can we create strongly correlated many-body systems of photons?
The central ingredient of correlated photonic systems is strong interactions between photons. Since photons do not interact in vacuum, inducing photon-photon interactions requires coupling light to a nonlinear medium. In the above figure, we ilustrate different regimes of nonlinear optical systems. In conventional materials (grey region), the effective interaction strength per photon is weak. This results in a classical mean-field nonlinearity, where quantum fluctuations are negligible and large photon numbers are required for interaction effects to be appreciable. On the other hand, small-scale quantum systems such as a single atom or optically active quantum dots exhibit strong nonlinearities at the level of a single photon. However, these systems have been severely limited by their lack of scalability.
In our team, we are trying to reach the regime of quantum many-body photonics, which will feature strong photon-photon interactions in a scaled up many-body setting. Such a scenario will set the stage for observing a vast array of completely new optical phenomena where photons exhibit emergent effects that have so far only been observed in matter-based systems!
Scalable quantum light sources
Photonic structures for confining light: cavities, integrated waveguides, photonic crystals.
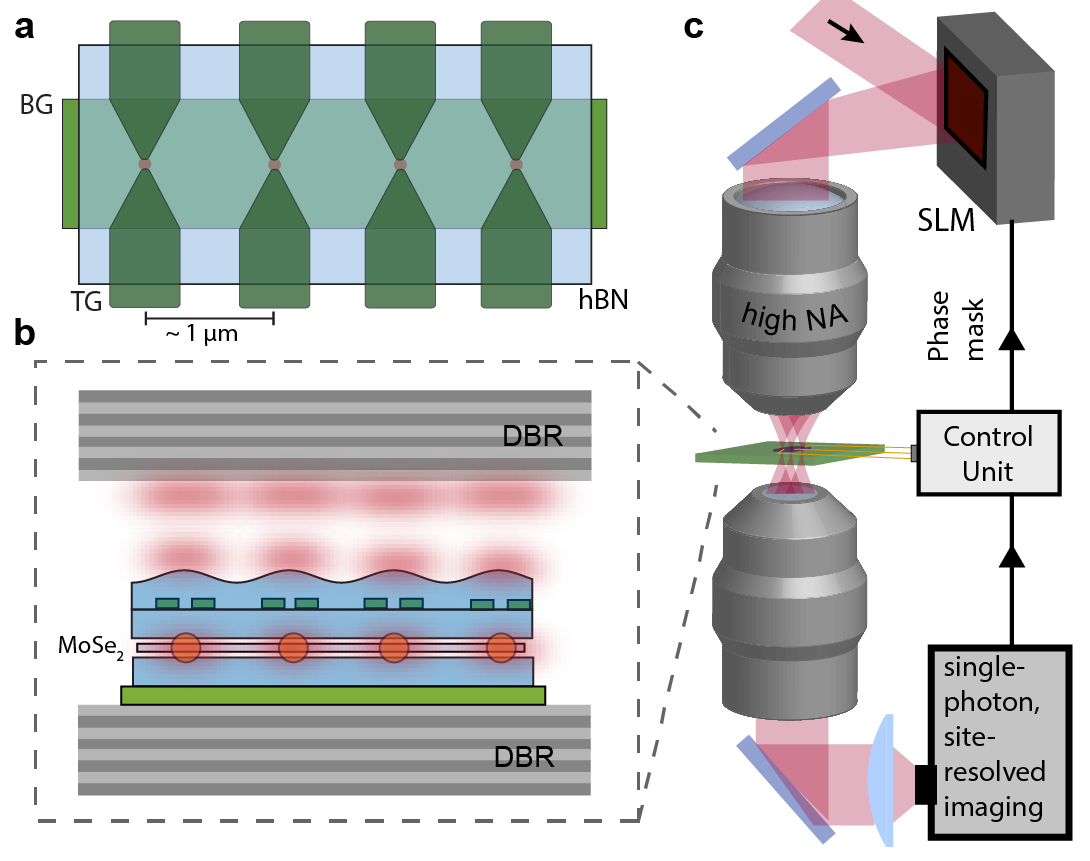
Photons are bosonic in nature, which means they like to bunch together. However, under very special conditions, photons can be made to arrive one at a time - a phenomena known as photon anti-bunching. This happens when light interacts with certain types of quantum emitters, which include atoms or molecules, Nitrogen vancancy centers, quantum dots etc. Such single photon sources are extremely useful for some applications, such as light-based quantum computing which uses single-photon states for performing quantum operations. One of the major challenges of using photons for such applications has been the availability of scalable single photon sources. Current approaches, which rely on chemical synthesis or material growth are limited in their scalability.
We are exploring ways to create quantum light sources, which are deterministic and scalable, and which can be incorporated into existing integrated photonics platforms. There are two main components in our approach: (a) create scalable quantum emitters in a solid state system, (b) integrate into photonics structures.
Deterministic Quantum Emitters
We are working on creating quantum emitters based on 2D materials that can be deterministically defined using gate electrodes. For this, our material of choice is two-dimensional van der Waals semiconductors, in particular Transition Metal dichalcogenides. These atomically thin semiconductors host optically excited particles, known as excitons which are bound pairs of a negatively charged electron and positively charged hole. Our approach relies on creating artificial quantum dots by confining these excitons to nanoscopic scales using electric fields. Our recent works have demonstrated this concept in different geometries, including 1D quantum wires and rings and zero-dimensional quantum dots.
Engineered photonic structures
The other major part of our research is in creating purpose-engineered photonic structures, such as optical Fabry-perto microcavities, dielectric waveguides and photonic crystal cavites. Our goal is to realize photonic structures which provide confinement of photons in tailor made geometries and very small mode volumes. To this end, we explore novel approaches such as Thermal scanning probe lithography, which allows us to create arbitrary potential landscapes for photons. By incorporating the quantum emitters into these photonic structures, we aim to realize scalable and deterministic single photon sources.